The Scanning Tunneling Microscrope
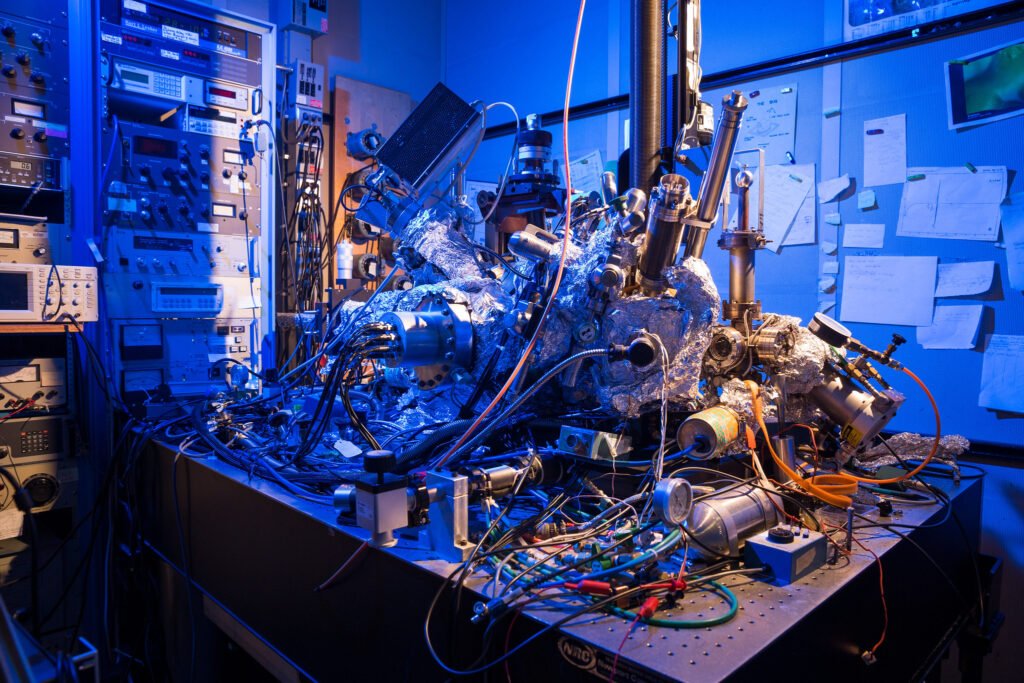
IBM Research. 2017. IBM Invented a Nobel-prize Winning Microscope to Store Data on a Single Atom. Flickr.
<https://www.flickr.com/photos/ibm_research_zurich/33346057445/in/photostream/>
Scanning Tunneling Microscope photographed at IBM Research Almaden campus in San Jose, California, on February 28, 2017. (Stan Olszewski for IBM)
The Nobel Prize in Physics 1986 was awarded jointly to Gerd Binnig and Heinrich Rohrer for their design of the scanning tunneling microscope. The official Nobel Prize announcement highlighted the significance of their work in advancing the precision of microscopy to the atomic level, opening up new possibilities for studying and manipulating materials at the nanoscale.
The basic principle of an STM involves scanning a sharp metal tip very close to the surface of a sample without making physical contact. The tip is brought within a few angstroms (1 angstrom = 0.1 nanometers) of the surface. When the tip is sufficiently close, electrons can “tunnel” between the tip and the surface. The tunneling current is highly sensitive to the distance between the tip and the surface, making it possible to map the surface topography with atomic precision.
Key features and components of a scanning tunneling microscope include:
Piezoelectric Elements: The tip and the sample are mounted on piezoelectric elements, allowing for precise movement in all three dimensions. This enables scanning of the tip across the surface.
Feedback Mechanism: A feedback loop maintains a constant tunneling current by adjusting the tip-sample distance. This feedback loop provides information about the surface topography.
Atomic-Scale Imaging: STM can produce detailed, real-space images of surfaces at the atomic level. It has been instrumental in the advancement of nanoscience by allowing scientists to study the arrangement of atoms on surfaces.
Manipulation: In addition to imaging, STM can be used to manipulate individual atoms. By controlling the tunneling current, researchers can move atoms around on a surface, opening up possibilities for nanoscale fabrication and manipulation.
Versatility: STM is applicable to a wide range of materials, including metals, semiconductors, and insulators. It is commonly used in physics, chemistry, and materials science research.
The scanning tunneling microscope has had a profound impact on various scientific disciplines, particularly in the fields of physics, chemistry, and materials science. It has become an indispensable tool for researchers working in nanotechnology, surface science, and condensed matter physics. The ability to visualize and manipulate individual atoms has paved the way for numerous discoveries and innovations in these fields.
source: https://en.wikipedia.org/wiki/Scanning_tunneling_microscope
How does and STM work?
Jubobroff. 2016. Scanning Tunneling Microscope. Wikipedia.
https://en.wikipedia.org/wiki/File:Scanning_Tunneling_Microscope.ogv
The invention of the Scanning Tunneling Microscope (STM) has significant implications for quantum mechanics, particularly in the context of understanding and manipulating matter at the nanoscale. Here are several ways in which the STM relates to quantum mechanics:
Quantum Tunneling: The working principle of the STM relies on a quantum mechanical phenomenon known as tunneling. Electrons, guided by quantum mechanics, can tunnel through a barrier even when classical physics would predict their confinement. In the case of the STM, electrons tunnel between the sharp metal tip and the sample surface, providing information about the local density of states and allowing imaging at the atomic scale.
Wave-Particle Duality: Quantum mechanics describes particles, such as electrons, as having both wave and particle-like properties. The STM’s ability to image and manipulate individual atoms is rooted in this wave-particle duality, where electrons exhibit both particle-like behavior (tunneling) and wave-like behavior (interference).
Quantum States of Atoms: The STM allows scientists to study and manipulate individual atoms on surfaces. This enables the exploration of quantum states of atoms, their electronic structures, and the interplay of quantum effects in confined systems.
Quantum Simulation: The STM provides a tool for the simulation and study of quantum effects at the atomic and nanoscale levels. Researchers can use the STM to investigate phenomena such as quantum confinement, quantum corrals, and quantum dots, gaining insights into the behavior of matter governed by quantum mechanics.
Manipulation of Quantum Systems: Beyond imaging, the STM has been used to manipulate individual atoms and molecules on surfaces. This level of control opens up possibilities for constructing quantum devices and exploring quantum information processing.
Quantum Coherence: The STM has been employed in experiments to investigate quantum coherence, where the quantum states of electrons can be maintained over extended periods. This is essential for understanding the dynamics of quantum systems and exploring potential applications in quantum computing.
In summary, the STM provides a tool to directly observe and manipulate matter at the quantum scale, bridging the gap between classical and quantum physics. It allows scientists to explore and exploit quantum mechanical phenomena in a controlled and systematic manner, contributing to both fundamental understanding and potential applications in quantum technologies.
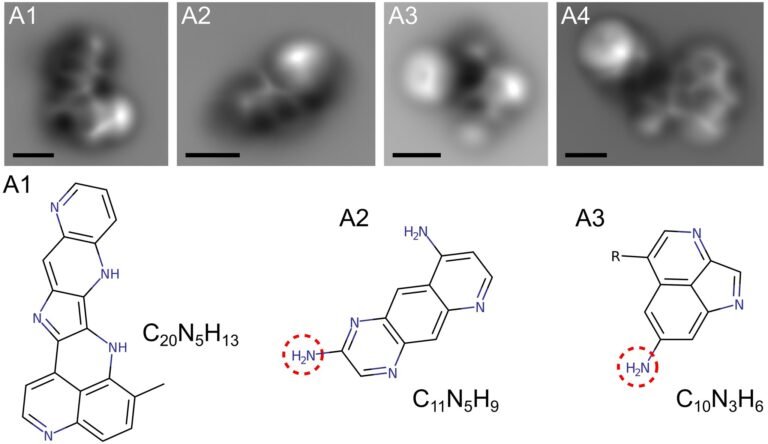
The pictures above show the visibility of the different atomic structures when viewed through an AFM microscope (see below). Credit: The Astrophysical Journal
IBM Research. 2021. Image 1. Flickr.
<https://www.flickr.com/photos/ibm_research_zurich/50908621241/>
Since the invention of the scanning tunneling microscope (STM) in 1981, various advancements and variations of the original STM design have been developed by researchers and companies. Additionally, new techniques and microscopy methods have emerged to complement and extend the capabilities of STM. Some notable developments include:
Atomic Force Microscopy (AFM): AFM is a scanning probe microscopy technique that operates on a similar principle to STM but uses a different feedback mechanism. It can provide high-resolution imaging of surfaces and is capable of imaging insulating materials, which can be a limitation for traditional STMs.
Scanning Tunneling Potentiometry (STP): This technique builds upon the STM principle but measures the local electrochemical potential of a sample. IBM researchers developed STP to study the electronic properties of materials at the nanoscale.
Non-Contact Atomic Force Microscopy (NC-AFM): NC-AFM is an advancement that eliminates the need for the tip to physically touch the surface. It operates in a non-contact mode, reducing potential damage to the sample and enabling imaging of delicate materials.
High-Speed STM: Researchers have developed high-speed STMs that can capture dynamic processes at the atomic level in real-time. This is valuable for studying reactions and behaviors that occur on very short timescales.
Combined Techniques: Some microscopy systems combine multiple techniques, such as STM with AFM or other spectroscopic methods, to provide a more comprehensive understanding of a material’s properties.
IBM, as well as other companies and research institutions, continue to contribute to the development of advanced scanning probe microscopy techniques. These advancements aim to enhance resolution, increase versatility, and improve the capabilities of these instruments for various applications in nanoscience, materials science, and beyond. The field of microscopy is dynamic, with ongoing research focused on pushing the boundaries of what is possible at the atomic and molecular scales.
Quantum Biology: Understanding What You're Made of
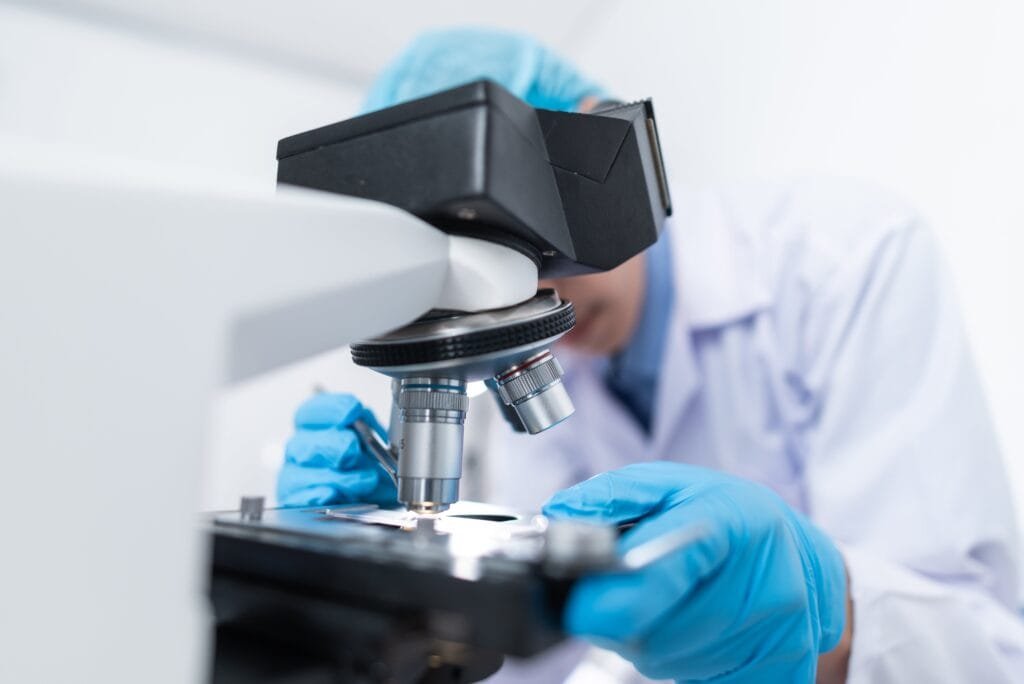
Notable works in the field include research by Huelga & Plenio (2013), who provide an overview of quantum biology’s fundamental principles, focusing on dynamics in quantum networks and their interaction with environments in biological contexts like photosynthesis and avian magnetoreception (Huelga & Plenio, 2013). Another important contribution is by McFadden & Al-Khalili (2018), tracing the origins of quantum biology back to early 20th-century quantum pioneers and highlighting recent findings that support the role of quantum mechanics in biological systems (McFadden & Al-Khalili, 2018).
Quantum biology suggests that despite the warm, wet, and noisy conditions within living organisms, which were previously thought to preclude quantum effects, nature has adapted to exploit these quantum properties, potentially revolutionizing our understanding of cellular functioning and offering new perspectives on the evolution of life (Goh, Tong, & Pusparajah, 2020).
In summary, quantum biology is a cutting-edge field that challenges traditional notions of biological processes, proposing that quantum mechanics plays a crucial role in various biological phenomena, thus opening new avenues for research and potential applications in medicine and technology.
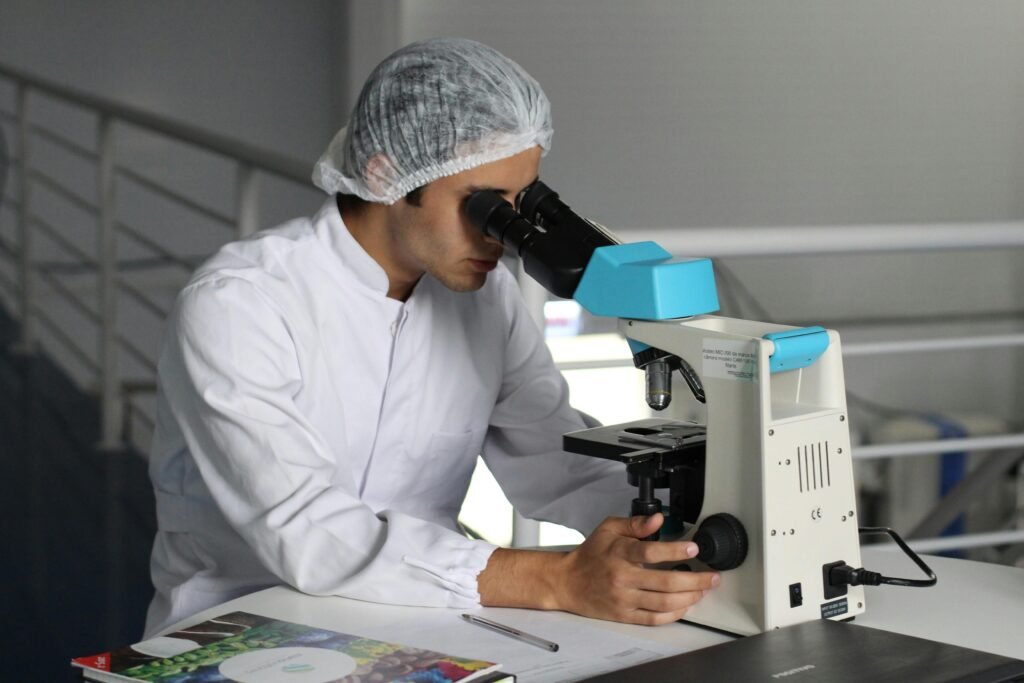